Fiber lasers are solid-state lasers that use optical fibers as an active gain medium. The different types are classified based on four factors: the laser source, the laser mode, the mode of operation, and the laser power. Fiber lasers can be valuable because of their highly efficient gain medium, a smart feedback loop through fiber Bragg gratings, and their robust optical cavity.
Fiber lasers find use in laser welding, cutting, etching, and cleaning. These devices are often seen in material processing and manufacturing, spectroscopy, electricity, transportation, medical applications, transportation, telecommunications, and military sectors. Fiber lasers can be more costly than other laser machines on the market, ranging anywhere from $20,000 to $200,000. However, their lower operating and maintenance costs help to offset the high price. This article will discuss fiber lasers’ benefits, design, uses, and application.
What Is a Fiber Laser?
A fiber laser can be defined as a solid-state laser that makes use of optical fibers as its active gain medium. A fiber made of glass silicate or phosphate absorbs unprocessed light from the pump laser diodes and converts it into a concentrated beam of a certain wavelength. The optical fiber is doped (incorporating a rare-earth element into the fiber) to make this possible. Different doping substances produce laser beams of differing wavelengths. Some popular doping elements include: dysprosium (2600-3400 nm), holmium (2025-2200 nm), and thulium (1900-2500 nm).
Fiber lasers are among the more energy-efficient laser options available, being up to 50% more energy efficient than CO2 lasers. There are several classification systems based on the: laser source, mode of operation, laser power, and mode (the size of the optical fiber's core, through which light flows).
Given the vast range of possible wavelengths, fiber lasers are ideal for a number of applications including laser cutting, cleaning, texturing, engraving, drilling, marking, and welding. This also makes it possible for fiber lasers to be used in many industries, including materials processing and manufacturing, spectroscopy, electricity generation, transportation, medical applications, transportation, telecommunications, and military hardware.
What Is the History of Fiber Laser Cutting?
Although the fiber laser was invented in 1961, the technology didn’t mature enough for commercial use until the 1990s. Most applications demand at least 20 W worth of laser power, whereas early fiber lasers could only output a few dozen milliwatts. Additionally, there was no way to produce high-quality pump light since early laser diodes were simply not efficient enough.
Who Invented the Fiber Laser?
The fiber laser was created in 1961 by Elias Snitzer and his colleagues at the American Optical plant in Southbridge Massachusetts, USA.
Snitzer's earlier research culminated in the creation of the first solid-state glass laser in 1961. His lab published both the first theory and observation of modes in an optical fiber. Both research programs contributed to the fiber laser’s development.
What Are the Advantages of a Fiber Laser?
In comparison to conventional laser heat sources, the fiber laser has a number of advantages as listed below:
- A fiber laser creates a beam inside the fiber, eliminating the need for a separate optical medium for beam delivery. It is therefore incredibly stable and simple to maintain.
- Fiber lasers offer extremely high optical gain. They’re capable of generating a kilowatt of continuous output power.
- Fiber lasers have a 30-50% power conversion rate compared to CO2 lasers' 10-15%, giving them a clear advantage in terms of energy efficiency.
- Fiber lasers are highly accurate even in complex designs because of the focused narrow beams. Since cutting laser devices do not physically contact the workpiece material and only a limited area is affected by heat, the cut remains clean and free of burrs, rough edges, or thermal deformation.
- The fiber's tiny core produces the beam. As a result, it is possible to create a high-quality, straight optical beam with less diffraction than with other methods.
- A fiber laser setup doesn't require routine maintenance like mirror replacement or adjustment. In contrast to CO2 lasers, fiber lasers have no blower or moving parts.
- Fiber lasers use less power and require less maintenance than others, which results in lower operating costs.
What Are the Disadvantages of a Fiber Laser?
Fiber lasers have some disadvantages; these are listed below:
- A fiber laser cutter typically has more expensive components.
- The delivery fiber cannot be separated from the source, making replacement a challenge.
What Are the Applications of a Fiber Laser?
Fiber lasers are useful in a variety of applications due to the many power levels they can produce. Among them are:
- Laser Marking: 1064 nm emission wavelength ytterbium-doped fiber lasers are considered ideal for laser marking applications. These lasers may leave sharp, durable imprints on plastic and metal surfaces. They can be customized to accommodate quick production cycles and can be manual or automated. Fiber laser equipment can be used to anneal, etch, and engrave as well.
- Laser Cleaning: Laser cleaning — the process of removing paint, oxide, and rust from metal surfaces — works best using fiber lasers. The procedure can be automated and customized for various manufacturing line conditions.
- Laser Welding: Fiber lasers play a significant role in the welding industry. In comparison to conventional methods, laser welding offers faster speeds, more precision, less distortion, higher quality, and greater efficiency.
- Laser Cutting: Fiber lasers have remarkably effective edge quality and can handle difficult cuts. They are ideal for items with tight tolerances. Due to the extensive list of advantages, fabricators have increasingly begun to favor fiber.
What Is the Maximum Power of a Fiber Laser?
The maximum power of a fiber laser can exceed 100 kW in some cases, particularly for fiber laser welding systems. Fabricators, however, don’t have 100 kW systems — their shop floors and beam delivery systems cannot handle that amount of power. Ultra-high power (UHP) fiber lasers are available in the range of 10 to 40 kW.
What Is the Average Power of a Fiber Laser?
The average power of a fiber laser depends on its application. The most popular types for marking applications are pulsed fiber lasers with low average power (10–20 W), pulse durations of 100 ns, and pulse energies in the range of 0.5–1.0 mJ. To optimize production cycles, high-speed ablation and surface cleaning require higher average power and pulse energy. To implement these cheap procedures, pulsed fiber lasers with more than 500 W of average power and 50 mJ of pulse energy have been produced. QCW (quasi-continuous wave) fiber lasers have been developed for long pulse ranges (second- to millisecond-pulse duration) to meet the requirement of high pulse energy (up to 60 J) at lower average power (a few reach the 100 W range).
How Does the Power of a Fiber Laser Scale?
Fiber lasers' ability to scale in power is constrained by Brillouin and Raman scattering as well as the short length of the lasers themselves. Many components, including amplifiers, switches, and logic elements, require nonlinear fiber configurations.
There are two classes of nonlinear effects in optical fibers. The first one is brought on by the Kerr effect, or the intensity dependence of the medium's refractive index. This phenomenon manifests as one of three effects, depending on the type of input signal: cross-phase modulation (CPM), self-phase modulation (SPM), or four-wave mixing (FWM).
The second nonlinear effect occurs when the optical field transfers some of its energy to the nonlinear medium via inelastic scattering. Such inelastic scattering can result in phenomena like stimulated Brillouin scattering (SBS) and stimulated Raman scattering (SRS).
Any form of stimulated scattering action can potentially be a source of gain for the fiber. In both processes, if the incident power rises above a specific threshold, the intensity of dispersed light increases exponentially. Because of the comparatively large frequency shift and the wider gain bandwidth, Raman amplification is more beneficial. The main distinction between them is that in Brillouin, the optical wave interacts with low-frequency acoustic phonons, whereas in Raman, the directed optical wave interacts with high-frequency optical phonons. Another key distinction is that SRS can happen in both directions while SBS only happens in the backward direction in optical fibers.
What Is the Beam Quality of Fiber Lasers?
A fiber laser's beam quality depends on both the strength of intracavity distortions and certain aspects of the resonator design. Ideally, the device would create what is known as a Gaussian beam, but actual beam quality is always imperfect. The mathematical expression for a perfect beam quality is M2=1. A well-focused laser beam concentrates more energy into a smaller space. Some processes like laser welding avoid perfect beam quality so that they don’t ablate much material. However, most (such as laser engraving and cleaning) require high-quality beams.
What Are the Alternatives to Fiber Lasers?
Some alternative cutting technologies to fiber lasers include:
- Gas/CO2 Lasers: A CO2 laser's wavelength is 10.6 mm. Compared to a fiber laser with the same power output, a CO2 laser has more energy to cut through thicker materials and can produce a smoother finish. Materials that can be cut with CO2 lasers include acrylic, leather, some plastics and foams, glass, paper-based materials, and wood.
- Crystal Laser Cutters: Crystal laser cutters operate at shorter wavelengths compared to CO2 lasers, meaning they have higher intensities, allowing them to cut through thicker and stronger materials. However, because of their high power, their parts deteriorate quickly. Crystal lasers are most often used to cut metals, ceramics, and plastics.
Which Materials Can a Fiber Laser Cut?
Fiber lasers can be used to cut the following materials:
- Metals such as: carbon steel, copper, brass, stainless steel, titanium, and aluminum.
- Plastic such as: acrylic, polyoxymethylene, and lucite.
- Graphite
On the other hand, fiber laser cutters are not suitable for: glass fiber, leather, ceramics, polycarbonate, ABS, HDPE, polystyrene, and polypropylene foam.
How Do I Know if the Power of a Fiber Laser is Sufficient for Laser Cutting?
As a general rule of thumb, materials below 0.1 inches won’t require more than 10 kW of output power. At 0.1 inch, it’s better to opt for a 15 kW machine. And at a thickness greater than 0.130, 20 kW or higher will suffice. Higher power results in more forgiving tolerances, faster cutting, and better accuracy. In general, doubling the power will result in a 20–30% rise in laser operating costs. However, increasing the laser power will also decrease the cost per inch and increase the laser machine’s capacity and cutting speed.
What Is the Reliability of a Fiber Laser?
There is no definitive answer to the question of how long a fiber laser will last. Like any device, fiber lasers can malfunction at any time from early life to the end of life. Across the industry, fiber lasers’ "mean time between failures" (MTBF) is roughly 100,000 hours. Note, though, that this will vary for various fiber laser types.
The MTBF is an estimate of the laser’s dependability and is calculated by testing several laser units, adding up the results, and dividing the result by the total number of failures.
How Are Fiber Lasers Designed?
Fiber lasers are designed based on specific design criteria. Each of the factors that affect laser design is discussed in the sections below.
1. Laser Cavity Design in Fiber Lasers
The laser cavity is where the gain medium is housed. It contains multiple optical elements that help to increase the strength of the laser. In the case of fiber lasers, the gain medium is a fiber optic augmented with rare-earth elements.
2. Fusion Splicing in Fiber Lasers
Fusion splicing is a technique of splicing fiber optic cables together so that light will pass through them unhindered. If properly fusion spliced, the laser will produce energy much more efficiently.
3. Laser Diodes in Fiber Lasers
Laser diodes are compact, effective semiconductors that transform electrical energy into laser light. These gadgets create the proper brightness and spectrum to be used to "pump" the doped fiber.
The rare earth ions embedded in the doped fiber then get excited by the laser beams produced by the laser diode pump sources. High gain levels are proportional to this excitement. The ability of a doping substance — such as ytterbium — to absorb the light from these pump lasers plays a role in its selection.
4. Dielectric Mirror in Fiber Laser
Dielectric mirrors are mirrors made up of more than one reflective material. They make the mirror more reflective than mirrors made from a single material. Fiber lasers use dielectric mirrors to further increase the gain of the laser output.
5. Distributed Feedback Lasers in Fiber Lasers
Distributed feedback (DF) fiber lasers have special qualities. A distributed feedback laser uses a gain medium and a periodic structure as its entire resonator, acting as a distributed reflector in the laser's operating wavelength range. A phase shift is often located in the center of this periodic structure. In essence, this structure functions as a series coupling of two Bragg gratings with optical gain.
The majority of distributed feedback lasers use a single resonator mode and are either fiber or semiconductor lasers. The distributed reflection in a fiber Bragg grating, which normally has a length of a few millimeters or centimeters, occurs in the case of a fiber laser.
6. Double-Clad Fibers in Fiber Lasers
Double-clad fiber is most common in high-power fiber lasers. The core of a double-clad fiber is doped with rare earth dopants. The desired beam quality attributes are provided by a standard single-mode fiber. However, single-mode pump laser diodes are necessary for a common single-mode fiber core. Properly designed, the diameter of this core allows for single-mode laser oscillation, which produces a high-quality beam.
7. Diffraction-Limited Beam Powers of Fiber Lasers
A diffraction-limited beam has the highest brightness, or radiance, for a given optical power. “Diffraction-limited” is used to describe a beam whose potential to focus on a small point for the given wavelength is only limited by unavoidable diffraction. In other words, it has the best possible beam quality.
The minimal beam parameter product is that of a Gaussian beam. It has flat wavefronts at the beam waist in addition to a Gaussian intensity profile if second moments are employed to define both values (beam focus). The diffraction limit does not apply to higher-order Hermite-Gaussian or Laguerre-Gaussian beams.
Given that its form is nearly Gaussian, a laser that works on the fundamental transverse resonator mode will typically have a diffraction-limited output. Intracavity beam distortions, such as those in the gain medium, can either deform the fundamental mode (though typically only with a mild effect on beam quality) or more frequently can result in transverse multimode operation, both of which can result in less-than-ideal beam quality. Optimizing the resonator design can be crucial for achieving a diffraction-limited output.
8. Stimulated Raman Scattering of Fiber Lasers
The nonlinear phenomenon known as stimulated Raman scattering occurs when energy from an optical pump beam is connected to longer wavelengths via the thermal phonons or vibrational modes of the medium's molecules. Any form of stimulated scattering action has the potential to be a source of gain for the fiber. If the incident power exceeds a specific threshold, the intensity of dispersed light increases exponentially at high power levels.
9. Stimulated Brillouin Scattering of Fiber Lasers
When narrow-band optical signals are amplified in a fiber amplifier or simply transmitted through a passive fiber, stimulated Brillouin scattering (SBS) frequently occurs.
10. Large Effective Mode Area in Fiber Lasers
The area that a waveguide or fiber mode covers in the transverse dimensions are quantified as the effective mode area. Optical fibers with very large effective mode areas (abbreviated as “LMA fibers”) are useful for various applications. However, they are frequently still guided in a single mode, or at best, have a few modes.
Large mode areas imply reduced optical intensities for a given optical power. These fibers have stronger damage thresholds and fewer nonlinear effects. Because of this, they can be used to amplify strong pulses or single-frequency signals in fiber amplifiers. Alternatively, in the case of passive fibers, they may even generate such light.
LMA fibers can reach area values of hundreds or even thousands of m2. Contrast this with ordinary single-mode fibers which have an effective mode area of less than 100 m2.
11. Saturable Absorber in Fiber Lasers
An optical component known as a saturable absorber has a specific absorption loss for light that decreases at high optical intensities. Saturable absorbers are mostly used in passive mode locking and for Q switching of lasers, which produces brief optical pulses. They can also be used in optical signal processing and nonlinear filtering outside the laser resonators to, for instance, clean up pulse forms.
12. Kerr Effect of Fiber Lasers
The Kerr effect is a nonlinear optical phenomenon that affects the intensity dependence of the medium's refractive index. It takes the form of one of three effects, depending on the type of input signal: self-phase modulation (SPM), cross-phase modulation (CPM), or four-wave mixing (FWM). Using the nonlinear optical Kerr phenomenon, Kerr-lens mode-locking (KLM) specifies the laser’s mode. With this technique, it is possible to produce light pulses with durations as brief as a few femtoseconds.
13. Semiconductor Saturable-Absorber Mirrors in Fiber Lasers
A semiconductor saturable-absorber mirror, or SESAM, is a particular kind of saturable absorber used in mode-locking lasers. A SESAM is a mirror structure that uses semiconductors as built-in saturable absorber media. SESAMs are frequently employed for laser passive mode locking, especially for solid-state bulk and fiber lasers.
14. Graphene Saturable Absorbers for Fiber Lasers
Owing to its unique optical properties like an ultrabroad tuning range, graphene, and its oxides are widely used as saturable absorbers for all kinds of fiber lasers. Light signals are absorbed by graphene-saturable absorbers in order to prepare for specific outputs to follow.
15. Dark Soliton in Fiber Lasers
A fundamental characteristic of fiber lasers with net normal dispersion is the generation of dark soliton, which is a dip in the amplitude of a continuous wave.
16. Multi-Wavelength of Fiber Lasers
Some fiber lasers can be used as multi-wavelength optical sources. This means they are capable of emitting light at multiple well-defined wavelengths at the same time.
How Does Mode Locking Work in Fiber Lasers?
A laser can emit pulses of light with an incredibly short duration, on the order of picoseconds (10-12 s) or femtoseconds (10-15 s), through a process called mode-locking. When a laser is locked in mode, one or sometimes two pulses are moving about in the laser resonator. A portion of the pulse's energy is released every time it strikes the output coupler mirror, resulting in a regular pulse train as the laser output. The pulse energy is refilled by the gain medium on each round trip.
The term "mode-locking" refers to a frequency domain interpretation in which a large number of axial resonator modes vibrate while their corresponding phases remain locked. The time domain, however, makes it simpler to understand what physically happens.
An actively mode-locked laser achieves mode locking using a modulator (such as an electro-optic type) that precisely synchronizes the resonator losses with the resonator round trips. When losses are at their lowest, the circulating pulse passes through the modulator. Slightly higher losses in the pulse wings cause the pulses to be briefer. A passively mode-locked laser's loss is modulated by a saturable absorber. The shorter the circulation pulses get, the quicker the loss modulation happens.
Passive mode locking produces a simpler laser setup since synchronization of the loss modulation is automatically completed and an electrical driver is not required. The pulse-producing process is more complex for a variety of reasons, making it, for example, far more difficult to achieve stable operation.
What Are the Main Types of Fiber Lasers?
The main types of fiber lasers are classified depending on the laser source (thulium-doped, erbium-doped, or ytterbium-doped), mode, mode of operation (multi-mode or single-mode), and laser power.
How To Classify Fiber Laser Types
The following can be used to classify fiber lasers:
1. Laser Source
The type of chemical used to dope the laser source impacts the light’s wavelength. Examples include thulium-doped, erbium-doped, and ytterbium-doped fiber lasers. The distinct wavelengths that each of these laser types produces allow them to be employed for different applications.
2. Mode of Operation
Differing laser configurations emit laser beams in different ways. For high peak strengths, laser beams may be "q-switched," "mode-locked," or "gain-switched." They can also be pulsed at a predetermined repetition rate (such as in pulsed lasers). Alternatively, if they were continuous (continuous wave fiber lasers), they might continuously transmit the same quantity of energy.
3. Mode
Mode describes the core size of an optical fiber, which is where the light travels. The two types of fiber laser modes are multi-mode and single-mode. Multi-mode laser core widths often range from 50 to 100 micrometers while single-mode laser core diameters typically come in between 8 and 9 micrometers. Single-mode lasers often provide better beams and more efficient laser light transmission.
4. Laser Power
The term "laser power" refers to the average power of the laser beam, which is given in watts. High-power lasers generate more energy and do so quicker than low-power lasers.
What Is a Fiber Laser System?
Every fiber laser system transmits light along an optical fiber cable consisting of silica glass. Because it is smaller and straighter than with other types of lasers, the resulting laser beam is more precise. Additionally, they are compact in design, exhibit outstanding electrical efficiency, require little maintenance, and can operate cheaply. They are frequently utilized in industrial settings to carry out cutting, marking, welding, cleaning, texturing, drilling, and other operations. This versatility comes from the various wavelengths they can produce. They are also used in other industries, like telecommunications and medicine.
What Is a Fiber Laser Machine?
A fiber laser machine is a device whose operation depends, at least in part, on a built-in fiber laser system. The core components of a fiber laser cutting machine, for example, include:
- Laser source
- Laser cutting head
- Servo motor
- Cooling device
- Air cutting system
- Laser host (bed, beam, table, and Z-axis system)
- Control system
- Stabilizer
What Are the Types of Fiber Laser Machines?
Table 1 lists some of the top fiber laser marker and engraving machines on the market:
Laser Machine | Power (W) | Material compatibility | Price ($) | Size |
---|---|---|---|---|
Laser Machine Omtech Fiber Laser Marker Engraver | Power (W) 20, 30, 50 | Material compatibility Metals, precious metals, brick and stone, hard plastics | Price ($) $3,099 | Size |
Laser Machine Cloudray AirMarker2 | Power (W) 20 | Material compatibility Metals and some hard plastics | Price ($) $2,100 | Size 110 x 110 mm |
Laser Machine Triumph 3D Fiber Laser Marking Machine | Power (W) 30, 50 | Material compatibility Metals, precious metals, laminates, foils, hard plastics, films, | Price ($) From $4,999 | Size 110 x 100 mm or 210 x 210 mm |
Laser Machine Dihorse Desktop Fiber Laser Marking Machine | Power (W) 20 | Material compatibility Most metals, some nonmetals | Price ($) From $4,769 | Size 110 x 110 mm up to 300 x 300 mm |
Laser Machine Ten-High Portable Fiber Laser Marking Machine | Power (W) 20-50 | Material compatibility Most metals some nonmetals | Price ($) $3,242 | Size — |
Which Industries Use Fiber Lasers?
The following are the top uses for fiber laser cutting equipment:
- Automotive sector
- Manufacturing of home appliances
- Fitness equipment manufacturing
- Manufacturing of lighting
- Manufacturing of decor and metal art
What Is the Working Principle of Fiber Lasers?
A fiber laser generates a sharp beam of light capable of effortlessly slicing through even some of the hardest materials. What sets it apart from other cutting devices and common laser cutters is the way energy is delivered and focused onto the workpiece. A laser, at its most basic, consists of three parts: gain medium, optical pump, and mirror array.
The pump produces the electrical energy that is converted into photons within the medium. That gain medium is the substance in which photons are produced and amplified. Finally, light is repeatedly reflected by the mirrors, resulting in the formation of a focused beam of light. By carefully controlling electromagnetic wavelengths, this method enables laser beams to transfer information with astounding accuracy and efficiency.
A fiber laser cutter uses a network of optical cables composed of fine fiber strands to transfer light waves straight into the workpiece. This gives the laser great precision and heat concentration, leading to cleaner cuts and stronger connections between materials.
This machine's software controller and the elements that assist and guide the material being cut are additional crucial pieces. Furthermore, fiber lasers can be tailored to suit particular requirements and applications by pairing them with specialized cutting heads.
How Does a Fiber Laser Work?
The following sections list the steps on how a fiber laser works:
1. Creating Light for Lasering
Electricity is converted by laser diodes into photons (light) which are then injected into the fiber optic. The diodes are also referred to as the "pump source" for this reason. Diodes use two oppositely charged semiconductors to produce light. The positively charged electrodes “desire” additional electrons while the negatively charged ones have spare electrons. In order to neutralize the charges, the free electron must jump between electrodes. As it does so, it releases a photon. The number of photons rapidly rises as the semiconductors are subjected to current.
2. Pumping Light Through Fiber-Optic Cables
The fiber core and the cladding are the two fundamental parts that fiber-optic cables utilize to concentrate light in one direction and produce a laser beam. The light travels through the fiber’s core, which is the only section of the cable that contains rare-earth metal and is constructed of silica glass.
3. Amplifying Light in Laser Cavity
When the laser diode light reaches the doped fiber, it interacts with the rare earth atoms, raising the electrons' energy level. This eventually results in a population inversion, which is required for the creation of a typical laser beam.
When a gain medium is in the condition known as "population inversion," more electrons than usual are in an excited state. They will release photons when they drop back to lower energy levels. Some of these electrons can only produce photons with a certain wavelength. When these photons come into contact with other excited electrons, they release comparable photons and drop to their lower energy states. This is the "stimulated emission" part of the acronym LASER (Light Amplification by Stimulated Emission of Radiation).
The incoming light from pump diodes re-excites the electrons as they return to their initial relaxed condition. A constant stream of unprocessed laser light results from the process, eventually reaching a balance between the stimulated and relaxed electrons.
4. Creating Laser Light of Targeted Wavelength
The doping component of the laser cavity affects the fiber’s output wavelength. This is crucial since various applications require different wavelengths. Erbium, thulium, ytterbium, neodymium, and other elements can be used as doping agents. Because each of these elements releases photons at specific energy states, different doping substances create different wavelengths. As a result, all photons produced by a given laser cavity will have the same wavelength. This is why each kind of fiber laser produces that wavelength and nothing else.
5. Shaping Laser Light
Different parts, such as lenses and beam expanders, can be employed to shape the laser beam as desired. Lenses are carefully chosen for different applications according to the advantages that they offer.
For applications where the laser penetrates the material — like laser texturing and engraving — short focal lenses are required. The short focal length concentrates more energy on a smaller region so that it can ablate material.
6. Releasing Laser Light
Finally, after the right lens has been selected, the laser is released to perform the action required. Fiber laser beams can be used to cut, weld, engrave, and clean certain materials (usually metals).
Which Laser Parameters are Utilized in Describing Fiber Lasers?
The parameters for fiber lasers differ between different laser modes. Laser power, beam diameter, and welding/cutting speed are the laser parameters usually associated with continuous wave lasers. The parameters used for pulsed lasers are pulse duration, energy, beam diameter, and repetition rate. Other parameters that are common with fiber lasers are wavelength, laser power, and beam quality.
What Are the Fiber Laser Operation Modes?
There are two main types of laser operation modes for fiber lasers:
- Continuous Wave (CW) Operation: A pump source keeps the laser's light output reasonably constant. This mode of operation, often known as free-running, is continuous in output. This is well-suited for purposes such as laser cutting and welding.
- Pulsed Operation: Light is released in a concentrated beam for a brief amount of time (a pulse). Pulses repeat at set intervals. This technique is frequently used to concentrate energy on a specific area of a substrate and to get the desired outcomes as soon as possible.
Which Mode of Fiber Laser is Better for Laser Cutting?
Continuous wave operating mode is better for cutting and welding because the power and intensity of the laser are constant throughout the cutting process, resulting in clean cuts. However, pulse operation is preferred for thicker metals due to its increased penetration efficiency — it requires less heat to achieve the same penetration depth.
What Is the Difference Between Fiber Lasers and CO2 Lasers?
The source from which the laser beam is produced is the primary distinction between fiber and CO2 lasers. In fiber lasers, silica glass combined with a rare-earth element serves as the laser source. In CO2 lasers, a combination of gasses, including carbon dioxide, serves as the laser source. Fiber lasers are classified as solid-state lasers while their CO2 counterparts are called gas-state lasers.
Summary
This article presented the fiber laser, explained what it is, and discussed the benefits and uses of a fiber laser. To learn more about fiber lasers, contact a Xometry representative.
Xometry provides a wide range of manufacturing capabilities, including sheet cutting and other value-added services for all of your prototyping and production needs. Visit our website to learn more or to request a free, no-obligation quote.
Disclaimer
The content appearing on this webpage is for informational purposes only. Xometry makes no representation or warranty of any kind, be it expressed or implied, as to the accuracy, completeness, or validity of the information. Any performance parameters, geometric tolerances, specific design features, quality and types of materials, or processes should not be inferred to represent what will be delivered by third-party suppliers or manufacturers through Xometry’s network. Buyers seeking quotes for parts are responsible for defining the specific requirements for those parts. Please refer to our terms and conditions for more information.
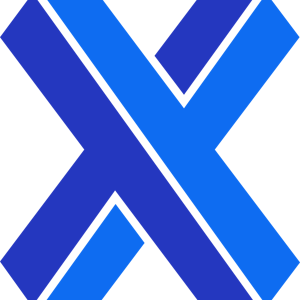